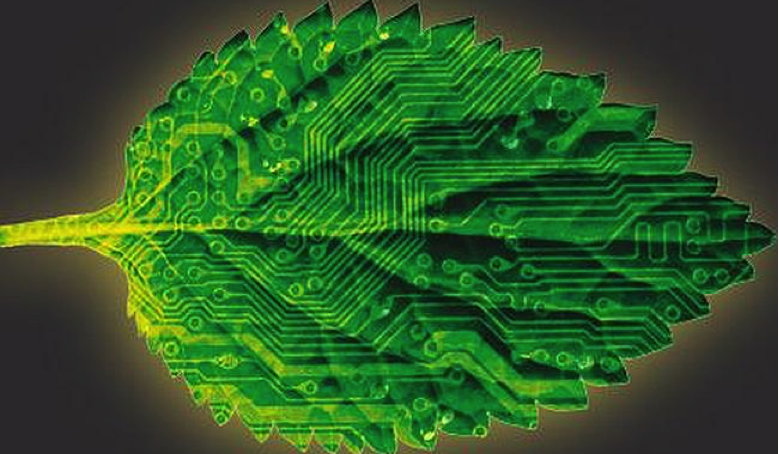
All this happens swiftly, in silence, at the temperature and pressure of the atmosphere, and gratis: dear colleagues, when we learn to do likewise we will be sicut Deus [like God], and we will have also solved the problem of hunger in the world. — Primo Levi
Ever since Antoine Lavoisier (1743-1794), the father of modern chemistry, discovered that so-called “fixed air” contains carbon and oxygen—carbon dioxide—scientists have labored to understand its properties and harness its potentials. It goes without saying that without CO2, life on this planet would simply not exist. But could it also be our undoing? Can too much of a good thing can be, well, too much of a good thing?
By some estimates, the amount of CO2 in our atmosphere has increased nearly 50% over the past fifty years. But it’s not all bad news. The rising levels of CO2 have delivered increased crop yields, improved water use efficiency, and enabled better quality of life for many around the world. These benefits, however, are also attended by a dark side: the increased acidification of the oceans, diminished air quality, and a receding of the polar ice caps, to name a few of carbon’s ill effects.
Whatever ones position on climate change, there are transcendent aspects to addressing this matter that are also intrinsic to the goals of generating sustainable energy sources and to improving the human condition. The efforts, technologies, and innovations dedicated to reducing CO2 levels to a carbon-neutral stasis promise wide-ranging benefits that go well beyond the battling of greenhouse gases. As such, this science has immeasurable and fundamental value to humanity.
The people at JCAP agree. JCAP is the Joint Center for Artificial Photosynthesis. It’s one of the Department of Energy’s “energy innovation hubs” focused on developing fundamental scientific foundations that can then be translated to the practical technologies needed to solve large-scale energy problems.
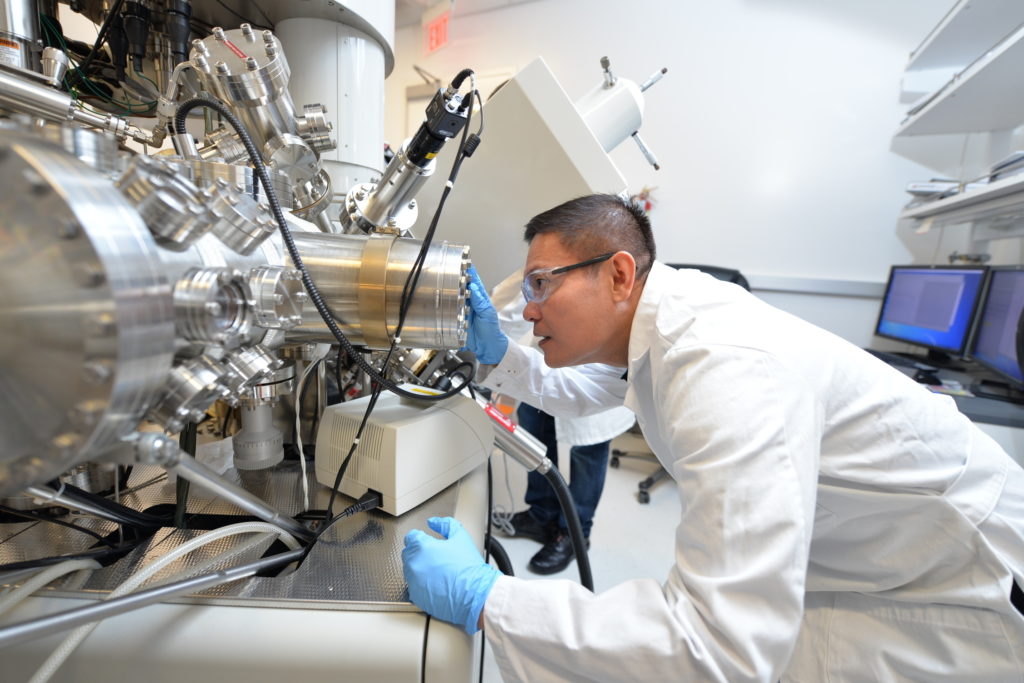
JCAP scientist, Dr. Jack Baricuatro, studying mechanisms of CO2 reductions reaction in the surface science laboratory in JCAP.
JCAP was founded in 2010 with an initial focus on water splitting—separating water into oxygen and hydrogen. In late 2015, the organization entered into its second phase: the very scientifically challenging and exiting goal of building scientific foundation through the discovery of new mechanisms, materials, and understanding of complex processes, for reduction of CO2.
Today, JCAP operates as a partnership among multiple institutions including California Institute of Technology, Lawrence Berkeley National Laboratory, UC Irvine, UC San Diego, and the Stanford Linear Accelerator Laboratory. Together, these organizations are rethinking the science that will affect the entire energy cycle. Dr. Ian Sharp, one of JCAP’s scientists, puts it this way: “Really, we’re tackling one of the most pressing challenges that faces humanity.”
But let’s back up a bit. Artificial photosynthesis?
This is actually not a new idea. The notion of artificial photosynthesis was suggested as early as 1912, by the Italian chemist Giacomo Ciamician. Driven by social concerns of the day, he proposed the abandonment of fossil fuels in favor of radiant energy provided by the sun and captured by photochemical devices. And in proselytizing on the idea, he assured his audiences that such a change would “not be harmful to the progress and to human happiness.”
Photosynthesis in nature, of course, relies upon a series of interactions beginning with the absorption of light. As a refresher, chlorophyll—from the Greek transliteration, “green leaf”—absorbs visible light in the blue portion of the electromagnetic spectrum (480 – 460nm), followed by the red portion (750 – 650nm). The green portions (530 – 490nm), however, are reflected, giving plants their familiar color.
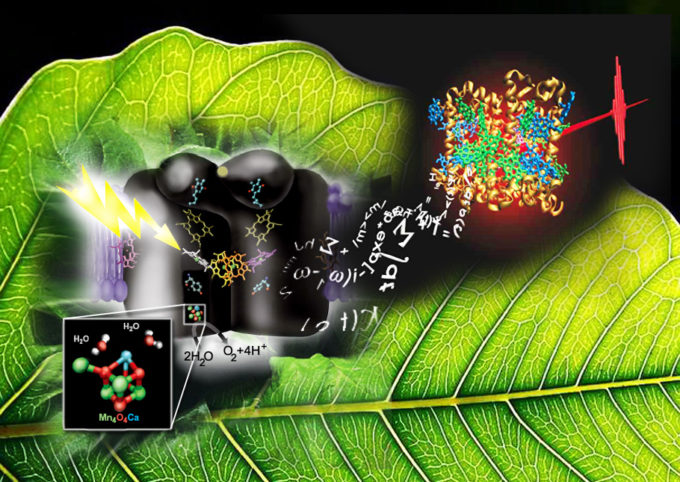
An impressionistic look at photosynthesis: at left, the oxygen-evolving complex in photosystem II (Yachandra/Yano lab); at right, electronic energy transfer in photosystem II’s light harvesting complex as simulated by supercomputers at NERSC, the National Energy Research Scientific Computing Center.
The process of photosynthesis, then, occurs as a light-dependent chemical reaction that first splits water into its constituent parts, then combines the resulting hydrogen with ambient carbon dioxide to produce glucose, expelling oxygen as the byproduct. Artificial photosynthesis draws its inspiration from these same processes, but uses them to generate fuels, like hydrogen and hydrocarbons.
There are two main areas of research in artificial photosynthesis: water splitting to generate hydrogen and CO2 reduction to make energy-dense fuels. The big idea behind artificial photosynthesis is that it allows for conversion of solar energy into chemical energy, i.e., storage of solar energy in chemical bonds. But generating enough fuel to power a society instead of a single plant poses some big challenges for scientists.
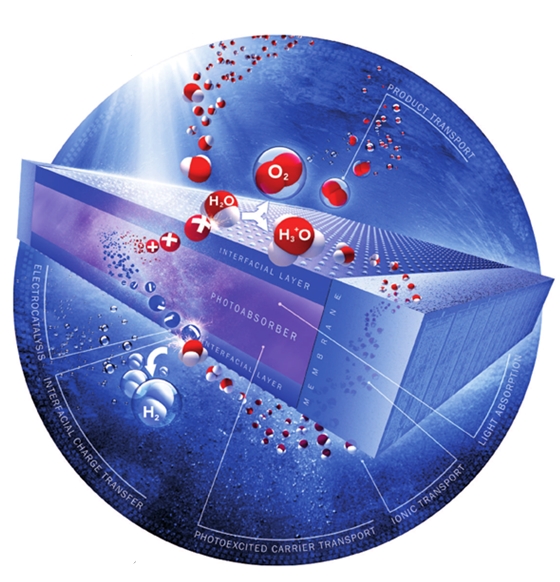
Solar-driven water splitting processes. Image courtesy of Angewandte Chemie.
An integrated cell for the solar-driven splitting of water consists of multiple functional components and couples various photoelectrochemical (PEC) processes at different length and time scales. The overall solar-to-hydrogen (STH) conversion efficiency of such a system depends on the performance and materials properties of the individual components as well as on the component integration, overall device architecture, and system operating conditions.
While hydrogen is abundant on earth, it is almost always found as part of another compound, e.g., H2O, and therefore it must be separated. There are numerous methods of splitting water, but they require energy, and so far, none of these methods is cost-effective when compared to hydrogen generation from fossil fuels. Cost reduction, therefore, is a primary technical challenge for renewable hydrogen production. Which brings us back to nature’s methods.
The imitation of the models and processes found in nature is called biomimicry, a phrase coined by Otto Schmitt, whose “Schmitt Trigger”—a staple of electronic circuits—emulates the system of nerve propagation—in squids. Artificial photosynthesis likewise takes a biomimetic approach in seeking to replicate a natural process in a controllable, efficient, and scalable way. But it isn’t easy. Scientists and technologists have struggled mightily to replicate nature’s ways of capturing, converting, and storing solar energy in ways that have economic value.
For example, the photovoltaic cell exploits solar energy, but it is a use-it-or-lose-it proposition: a cost-effective way of storing the electricity the cells produce remains a substantial challenge. In photosynthesis, the plant actually locks solar energy within the chemical bonds in the glucose molecule it creates.
Now, remarkable as this process is, even if we were able to fully tap the power of photosynthesis, it still wouldn’t be good enough to satisfy the economic drivers for large-scale energy production. While the plant might be happy enough, it turns out that natural photosynthesis is not terribly efficient. In fact, it is quite inefficient: of the total solar spectrum that is incident upon the leaf, nearly half falls outside chlorophyll’s active range of 400 – 700 nm. And of the in-range photons, nearly a third are lost to incomplete absorption; not all photons score a hit on the chloroplasts. When all such degradations are accounted for—and there are several other factors—we end up with something on the order of 1% – 3% efficiency. Not exactly the stuff of commercial grade scalability. The minimum solar-to-chemical conversion efficiency required for commercial viability is closer to 10%.
The challenge, then, is to improve upon the nature’s process, which means absorbing more light at a wider range of wavelengths, ideally across the entire solar spectrum. That is precisely what the researchers at JCAP are doing. And they’re making tremendous progress.
Professor Harry Atwater and JCAP’s director, explains “JCAP, over the last five years, has had a mission to develop a solar fuels generator that was more efficient than natural photosynthesis. I’m happy to say that in producing a 10% solar-driven device, it was able to accomplish its goals. It’s these scientific advances that will eventually bring about a renewable carbon cycle for our economy. And to that end, we are in the second year of our five-year CO2 reduction project, in which we will parlay these advances.”
And the excitement at JCAP is palpable. “What’s really exciting,” says Dr. Adam Weber “is the fact that we can produce something, in an ideal world, just from the air; from the sunlight that’s hitting the earth, we can produce something that can feed into your car. No longer do we have to think about digging up oil.”
To accomplish that work Dr. Charles McCrory, a JCAP alum currently working at University of Michigan explains, “First, we develop light absorbers to capture solar energy. Second, we develop catalysts to facilitate the chemical reactions, and lastly, we develop membranes to separate the resulting chemical fuels from the oxygen which we’re also producing at the same time.”
The catalysts can be classified into two general categories. Dr. Ian Sharp explains, “One of these is heterogeneous catalysts which are made from solid materials like metals and oxides of metals. The other would be molecular catalysts. These molecular catalysts are ones that can be finely tuned by chemists to mimic natural processes. These molecules can support reactions such as the combining of two protons and two electrons to form hydrogen gas. For the more complex fuels that we would like to generate in the future, such as methane and methanol, these catalysts have to have very complex configurations to enable each and every step of that reaction to be done with the highest degree of efficiency and minimize the energy that we have to input.” the current focus of JCAP is on the development of heterogeneous catalysts for CO2 reduction and its “sister” oxidation reaction – oxygen evolution reaction.
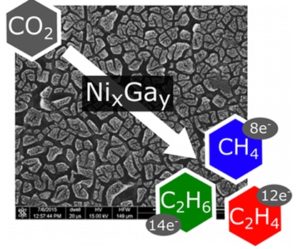
Electrochemical reduction of CO2 by bimetallic alloys. Image courtesy of American Chemical Society.
Not surprisingly, JCAP is also involved in materials discovery. “By the discovery of new materials,” Dr. Sharp says, “we’re enabling new technologies that no one predicted.”
Dr. John Gregoire explains, “A lot of materials absorb sunlight, but we need a material that not only absorbs it, but also harnesses that solar energy to perform specific chemical reactions. And we need materials that can do this all at once so that we can integrate those materials into a high-efficiency device. If we can discover the right set of materials and successfully integrate them, we’re really creating the ultimate renewable technology. But with that great promise comes the great challenge of finding these materials that are not presently known.”
Another constraint is that these materials have to be very inexpensive and easily obtained in large quantities. “And currently,” Gregoire notes, “our only source for elements is the Earth. So we need earth-abundant materials to include in this device to make it scalable and manufacturable.”
Gregoire explains that over the decades of solar fuels research, practically every element from the periodic table has been considered, but none of them are good enough. “That means we need to combine the elements in different ways that no one has ever done before. To make and test all those combinations, you need very, very fast experiments.” And for that reason, JCAP is built for speed.
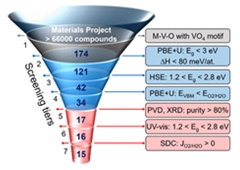
High-throughput theory and experimental discovery of new materials. Image courtesy of National Academy of Sciences.
It turns out that JCAP is not alone in this endeavor: there are thousands of researchers the world over working to crack this grand challenge. JCAP is joined in this global effort by the Max Planck Institute in Germany, the Ministry of Education, Culture, Sports, Science and Technology (MEXT) in Japan, and the Korea Center for Artificial Photosynthesis (KCAP), among others—all of whom are making gains in artificial photosynthesis research. Additionally, the $20M NRG COSIA Carbon XPRIZE will “challenge the world to reimagine what we can do with CO2 emissions by incentivizing and accelerating the development of technologies that convert CO2 into valuable products.” That’s a lot of activity.
“And hence,” Gregoire says, “we have a high-throughput experimentation group to do that discovery work. We work with our colleagues to generate ideas faster than traditional experiments can test them. And then we can invent instruments and invent techniques to perform those experiments a hundred to a thousand times faster than traditional methods.”
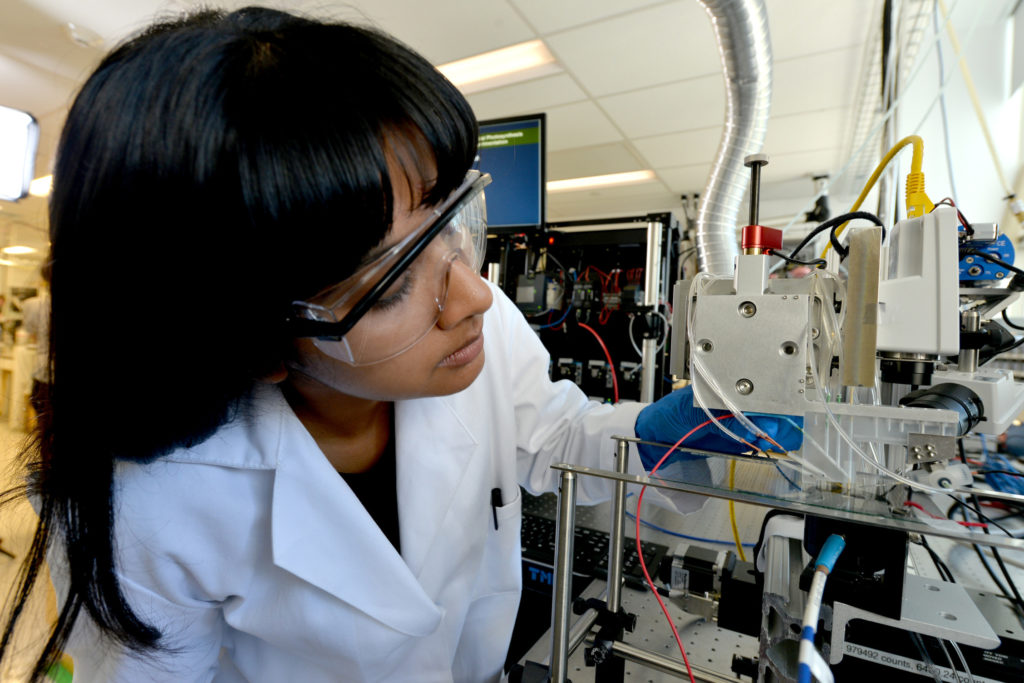
JCAP scientist, Dr. Aniketa Shinde, performing fast screening tests of the new materials in the high-throughput laboratory in JCAP.
In the end, JCAP is accelerating a development process that spans the discovery phase to a translational phase to an operational device phase from many months to a matter of weeks. This is not only enabled by the high throughput techniques but also the interdisciplinary nature of the energy innovation hubs where scientists and engineers work together to translate discoveries to device prototypes. Gregoire adds, “When we invent the tools, we get to synthesize materials that no one in the world has ever synthesized before. So being able to discover new things every day is really exciting.”
Some of the hallmark technological achievements of JCAP include the synthesis of high performance materials using a scalable technique based on inkjet printing, the optimal integration of the materials so the device works even better than the sum of its parts, and the establishment of comprehensive device modellings to guide the development of solar fuels prototypes.
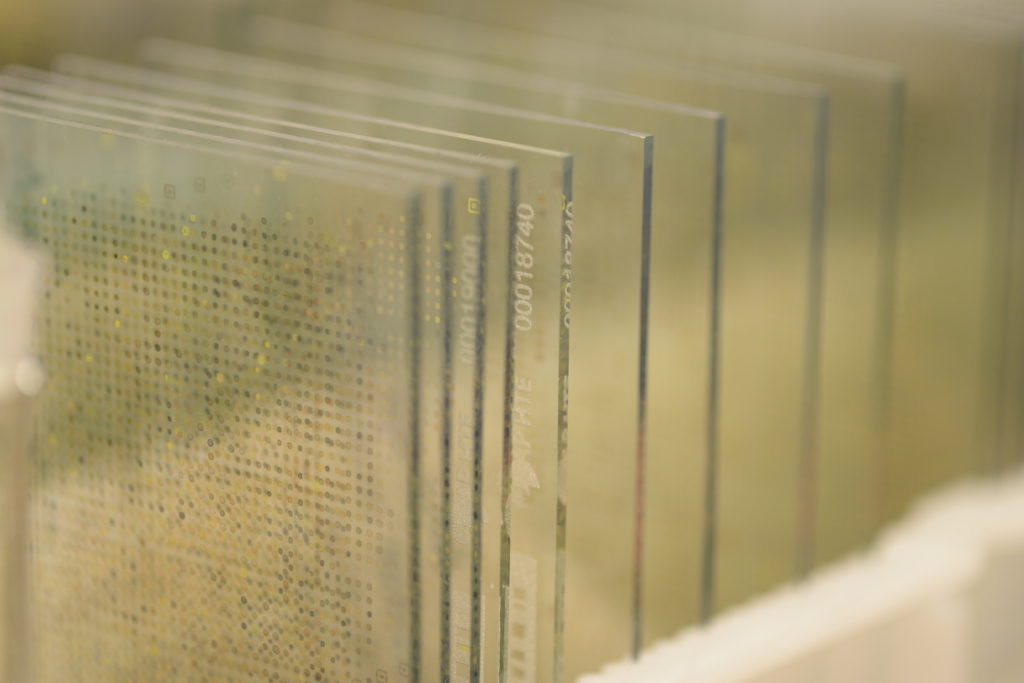
Inkjet-printed libraries of new materials.
Indeed, a key part of the technology development is prototyping these new devices and benchmarking their performance levels to determine how active each of the new components are. Dr. Charles McCrory explains that JCAP’s unique catalyst benchmarking process yields more reliable results. “There are thousands of researchers working on this problem, and each one is working under slightly different conditions. What we do is make apples to apples comparisons of all the different catalysts that are being developed throughout the world. We study the catalysis and the activity under the exactly same conditions. That’s important, because if you’re trying to determine how fast a car can go, the track conditions matter. So we’re testing the speed of each car on the exact same track.”
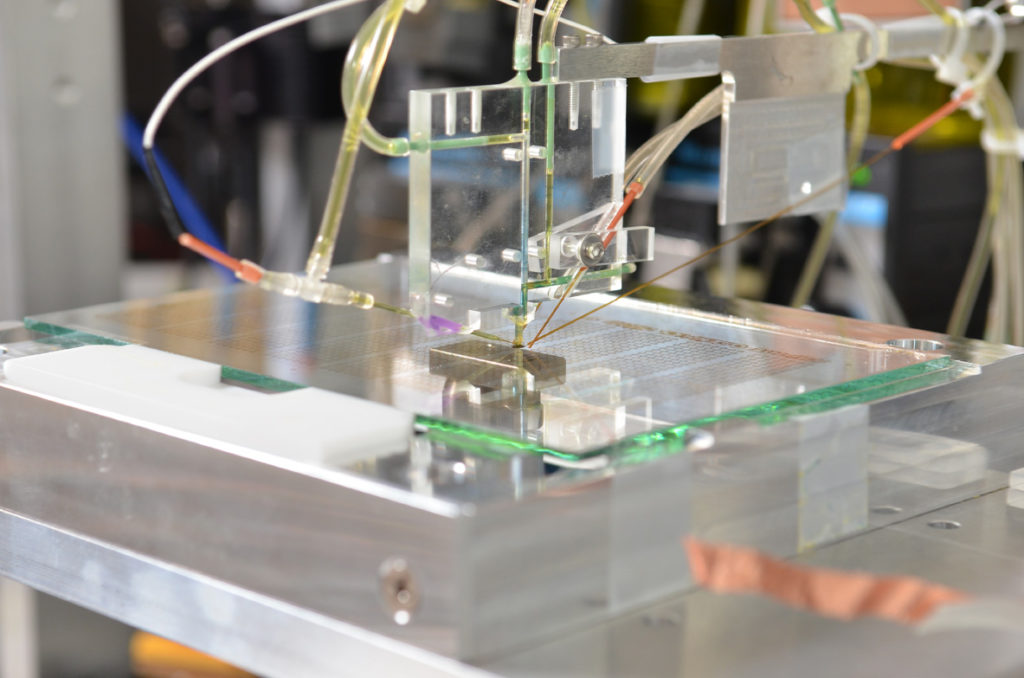
Rapid screening of inkjet-printed libraries of materials.
JCAP’s rapid pace of progress can be attributed to its organizational principles. JCAP is a multidisciplinary hub that is designed to efficiently cross-pollinate know-how across its four research thrusts: electrocatalysis, photoelectrocatalysis, integration of materials, and test bed prototypes and modeling. As Dr. McCrory attests, “One of the best things about working at JCAP is you get to work with people from the wide spectrum of backgrounds—physicists, chemists, chemical engineers, mechanical engineers. And you can leverage off their expertise, as well as the main facilities we have here to solve problems in unique ways.”
JCAP’s efforts to take artificial photosynthesis from the lab to practical devices that can be used for the large scale production of energy hold tremendous promise. Professor Atwater, sums it up: “The ability to bridge the divide between systems that operate on a very small scale to technologies that can be scaled up into large systems for generating fuel from sunlight, will ultimately allow us to produce the energy we need—and do so in a carbon neutral way.”